Aqueous Interfaces
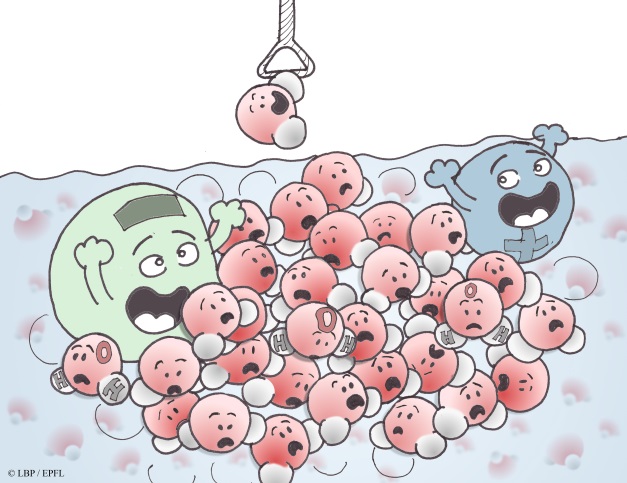
Nano- and micro-scale aqueous interfaces
Water. No substance on earth is so intimately linked to our well-being. Without it, we die. On a more scientific level, without water, membranes -the structures that provide the architecture of our cells and organelles- cannot function. Charges and charged groups cannot be dissolved, self-assembly cannot occur, and proteins cannot fold. Apart from the intimate link with life, water also shapes the earth and our climate. Our landscape is formed by slow eroding/dissolving processes of rocks in river and sea water; aerosols and rain drops provide a means of transport of water. Our society depends on products that all relate to water and aqueous systems, such as food products, medicine, and consumer goods.
In most of the above mentioned systems it is the interfacial region (of the membrane, the droplet, or the particle) that determines much of the physical, chemical, biological, and geological properties. Interfacial water is often considered in one of two ways: As a background, describable by a single parameter, or simply omitted. Alternatively, it is studied in great detail in an environment or condition that is precisely defined but so oversimplified that it has little to do with the real world. Aqueous interfaces are mostly studied in vacuo, or as a planar water/air interface.
However, interfacial water exists on different length scales, from sub-nanometer to micron sized (corrugations, organelles, membranes, liposomes), and is often buried inside another solid or liquid environment that is not at all comparable to vacuum or air. It can also be subject to conditions of flow or electrostatic fields. The absence of molecular knowledge of realistic interfaces is due to a lack of tools that can access buried nano- or microscopic interfaces in liquids and solids. Research at LBP is aimed to develop non-invasive label-free optical tools that can probe aqueous systems and interfaces on different length scales and to use these tools to understand the molecular level properties of water in diverse systems such as in solutions, at buried interfaces, in and outside droplets, and curved nanoscale membranes (illustrated in the below Figure).
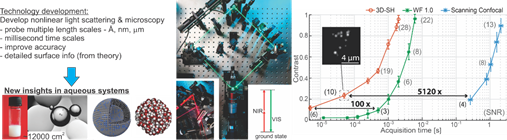
The LBP approach to study aqueous systems with a multiscale toolbox. Left: Rationale of our work, and illustrations of the studied systems. Middle: Photos of parts of the SFS systems (left inset), the first SHG wide field microscope (WF 1.0), and an optical scheme for SHS (right inset). For SFS the excitation is done with an infrared pulse at resonance with a vibrational mode and a non-resonant visible pulse. Right: Throughput curves for SH imaging of 50 nm BaTiO3 particles on a glass cover slip, measured with our homebuilt wide field systems “3D-SH’, ‘WF 1.0’ and a commercial device (‘scanning confocal’ Leica SP5, EPFL microscopy facility).
A variety of systems have been studied, comprised of most notably, emulsions (oil droplets in water and water droplets in oil) and membrane interfaces of liposomes. We find in most studies that the length scale of the system determines interfacial structure and that the chemical interfacial details drive the outcome of many processes.
Highlights:
ACS Nano, (2017) 111, 12111-12120
Nat. Commun., (2017), 8, 15548-1-6
Chem. Phys. Lett. Frontiers, (2014), 615, 124-130
Angew. Chem. Int. Ed., (2014), 53, 9560-9563
J. Am. Chem. Soc. (2010), 132, 2122 – 2123
Nanoemulsion
Nanoemulsions of ~100 nm oil droplets in water are stabilized by dilute monolayers of charged surfactants rather than dense monolayers as is the case in planar systems made of the same components (see below figure). The interfacial molecular conformation of the constituents is also different. The cause of this difference appears to be a different balance of molecular interactions, and most notably charge-charge interactions (which carry over several microns in low dielectrics, but not in the aqueous phase). Furthermore, positively charged droplets are less stable than negatively charged droplets, as caused by a different balance between hydrogen bond interactions (they only form with negative charges and not with positive charges). As a consequence, we speculate that this is why biological interfaces are primarily neutral or negatively charged. In addition, the distribution of ions in the interfacial region, and the impact it has on interfacial structure was investigated by probing all ionic species involved using their vibrational resonances and the water using its second harmonic signature. This approach differs from the more commonly performed studies of probing the influence of ions on the water structure at extended planar air/water interfaces. It shows that also in this case the balance of interactions is delicate (hydrophobic, charge-charge and hydrogen bonding) and small changes therein can dramatically change the structure of the interface. In nanoscale systems the electric double layer is more complex than simple mean field models suggest.
In our studies we repeatedly find that the balance of interactions dictates the stability, the interfacial structure, and reactivity of nano- and micron sized oil droplets in water, and this depends on the length scale of the systems in ways that have not been considered thus far. With the currently renewed interest in such systems as reaction vessels in flow systems and their importance in a multitude of biotechnological and food products, and as model systems our work in this area will provide a benchmark in understanding the molecular mechanisms that dictate macroscopic function.
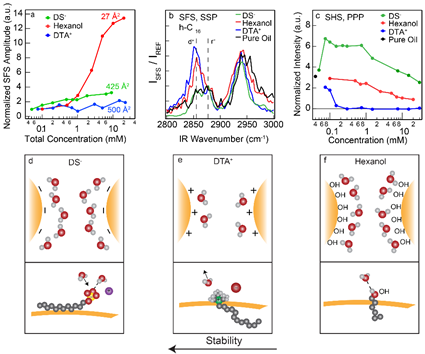
The molecular mechanism of nanodroplet stability. Top: SFS amplitudes reflecting surfactant densities (a), SFS spectra of interfacial oil (b), and the orientational order of interfacial water (c) for negatively charged, positively charged and neutral surfactants. Instead of all behaving in the same way as dictated by the commonly accepted geometrical packing model, there are clear differences in the surface structure and droplet stability (not shown). Bottom: Illustration of stability mechanism (top) and molecular interfacial structures (bottom) for three representative, negative (d), positive (e) and neutral (f) surfactants. From: ACS Nano, (2017) 111, 12111-12120 (PDF)
More information
ACS Nano, (2017) 111, 12111-12120
J. Chem. Phys. (2016) 145, 044706
J. Phys. Chem. C, (2015), 119 (31), 17725–17734
J. Am. Chem. Soc. (2014), 136, 2040 – 2047
Angew. Chem. Int. Ed., (2014), 53, 9560-9563
J. Am. Chem. Soc. (2010), 132, 2122 – 2123
Hydrophobicity
One question that repeatedly arises in studying hydrophobic nanoscale systems regards the nature of hydrophobic interactions and the stability and structure of oil water interfaces. At this moment there is a large body of scientific work that appears to support contradicting views. Is the hydrophobic interface positively charged, negatively charged or neutral? Are there H+, or OH– ions present? We have so far not detected any of these surface species. Our work suggests the importance of charge and charge transfer effects at aqueous interfaces but future work will hopefully solve this matter that lies at the heart of explaining the structure of biological systems. The below figure sketches to different hydrophobic interfacial structures that will have very different SF spectra.
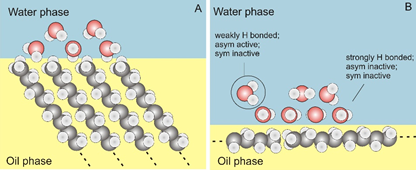
Schematic illustrations of possible hydrophobic surface structures. (A) An ordered structure of alkane chains in contact with water, as commonly present in a perfectly crystalline self-assembled monolayer. Water molecules that have their dangling OH bonds in the cavities formed by the methyl groups are sketched. (B) An illustration of an alkane/water surface with more parallel oriented alkane molecules. Here, there is much less space for dangling OH bonds that have their vibrational coordinate directed along the surface normal. Such different structures result in different SF spectra.
More Information:
Chem. Rev. (2016) 116, 7642-7672
Chem. Phys. Lett. Frontiers, (2014), 615, 124-130
Angew. Chem. Int. Ed. (2012), 51, 12938 – 12940
J. Am. Chem. Soc. (2011), 133, 10204 – 10210
Water droplets
Water droplets are part of the atmosphere, the earth and many other systems. In such systems the interfacial region determines the chemical properties of the drops. But what is the structure of a water droplet interface? We have studied the surface structure of water using sum frequency scattering and find that water droplets in hydrophobic liquids of approximately the same size have a surface structure that contains more ordered water than equivalent planar interfaces made of identical components equivalent to a reduction of temperature by ~50 K, suggesting that the reactivity of such interfaces is less than expected. This means that atmospheric processes probably occur at very different speeds than is currently being factored into climate models.
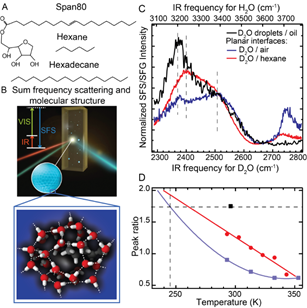
Surface structure of water droplets. A: Chemical structure of hexadecane, hexane and Span80 B. Illustration of a vibrational sum frequency scattering experiment. A femtosecond infrared (IR) and a picosecond visible (VIS) laser beam overlap in space and time in the sample and generate scattered sum frequency light from the water droplets. The inset illustrates the surface hydrogen-bond structure of a water droplet embedded in a hydrophobic liquid. In this figure we sketch a possible molecular arrangement of the water molecules at the surface of a droplet surrounding an alkane tail that protrudes into the water phase (looking from the inside of the droplet). C SFS spectra of D2O droplets in d34-hexadecane with 5 mM Span80 (black) and reflection SFG spectra of the planar D2O/air interface (blue) and planar D2O/hexane interface (red). D. The ratio between the low and high frequency bands of the SFG spectrum of the planar water/air and water/hexane interface (blue and red markers) and an extrapolation with a quadratic polynomial fit to a lower temperature range (blue and red lines). The ratio for the room temperature water nanodroplet spectrum is shown as a black marker.
More Information:
Nat. Commun., (2017), 8, 15548-1-6
Electric double layer structure
The electric double layer is a layer that surrounds any aqueous charged interface and is thus a determining factor in interface stability and nearly all forms of biochemical change. Although the layer is usually modelled with a mean field continuum model (meaning that all ions behave as point charges and that water is a passive dielectric), a plethora of specific effects that indicate the opposite are known. These effects have not been quantified, however. We have started to do this with our multiscale toolbox and find that the nanometer length scale and the hydrophobicity of the cations is crucial in determining whether the ions only influence the water structure in the interfacial region or also the surface itself. In addition, the ability to determine the average value of the surface potential is a great help.
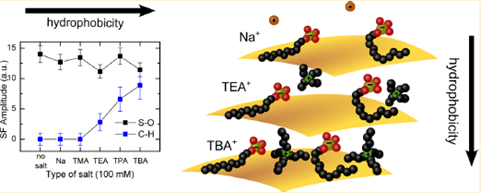
The surface presence of negative and positive ions does not only depend on charge but also on the hydrophobicity of the ions.
More Information:
Chem. Rev. (2016) 116, 7626–7641
J. Am. Chem. Soc. (2013), 135, 19330 – 19335